During the past two decades, there have been tremendous developments in near-field imaging and local probing techniques. Examples are the Scanning Tunneling Microscope (STM), Atomic Force Microscope (AFM), Near-field Scanning Optical Microscope (NSOM), Photon Scanning Tunneling Microscope (PSTM), and Scanning Thermal Microscope (SThM). Today, the Scanning Probe Microscopy (SPM) family has become ubiquitous for applications from surface topography and surface modification nanoscale thermal imaging, to bio-molecular sensing, atomic-level detection, and nanolithography. Near-field radiation may play a significant role in the heat transfer process between the SPM cantilever tip and the surface over which it scans, due to photon tunneling through evanescent modes and surface-waves (such as plasmon or phonon polaritons). Furthermore, the radiative properties of nanostructured materials may differ significantly from their bulk properties. Although the existence of near-field radiation has been known for over 100 years, experimental validation is required to realize the opportunities with near-field radiation at the nanometer length scale. The purpose of this research is to investigate nanoscale thermal radiation both theoretically and experimentally.
To better understand micro/nanoscale thermal radiation, a theoretical study on the direction of the energy flow was performed by examining the group velocity upon refraction [1]. The surface polariton phenomenon was analyzed to study its impact on the radiative properties [2]. More recently, we have performed a comprehensive investigation of the radiation energy transfer between two semi-infinite parallel plates at different temperatures, involving silicon with varying dopant concentrations, when the distance of separation down to 1 nm [3]. The net radiation heat flux is calculated by means of the fluctuational electrodynamics. A Drude model that considers the effects of temperature and dopant concentration on the free carrier concentrations and scattering times is adopted, after a careful parameter selection and comparison with existing optical property data. The calculated results show that the dopant concentration strongly affects the radiation heat flux when the two media are separated at nanometer distances. Enormous enhancement in nanoscale radiation heat transfer has been found for heavily doped silicon. For heavily doped silicon plates separated at a distance of 1 nm, the present study predicts a radiation energy flux of over five orders of magnitude greater than that between two blackbodies placed far apart. At the separation distance of 1 nm, the net energy flux can exceed 1 GW/m2 with (n-typed) silicon emitter at 1000 K and receiver at 300 K, for dopant concentrations of 1021 cm-3. The calculated heat flux is 10 times that of air conduction at atmospheric pressure. The radiation heat transfer coefficient can be 1 MW/m2×K at 1 nm and 10 kW/m2×K at 10 nm separation distance. The theoretical understanding gained from the present research will facilitate the design of experiments that utilize near-field radiation to enhance heating or cooling at the nanoscale for applications such as thermal control in nanoelectronics, energy conversion, and nanothermal probing and manufacturing.
Figure 1 shows the predicted nanoscale radiation heat transfer between two n-type phosphorus-doped silicon plates, separated by a distance L in a vacuum. In the far-field, the next radiative flux is limited by the emissivity of silicon surfaces and is about half of that between two black bodies. As L becomes smaller than 1 mm or so, the near-field effect becomes important and the net heat flux exceeds that between two blackbodies (in the far-field). For two intrinsic silicon media, the near-field heat flux saturates at about 100 nm, i.e., further reducing L does not increase the heat flux. The enhancement is limited to n2, where n is the refractive index of silicon. When both the emitter and receiver are heavily doped, the enhancement is much greater in the near field that does not saturate at L approaches zero. When both media are doped, the radiative heat flux can be enhanced more than 10,000 times as compared to the far-field heat flux between silicon and silicon [3].
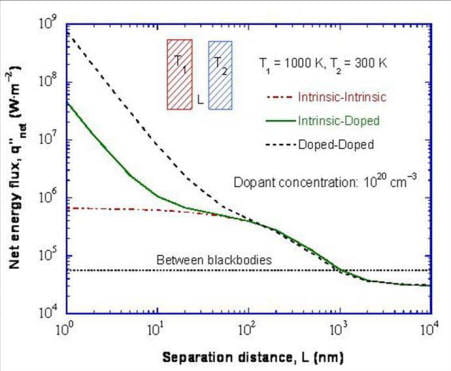
Figure 1. Predicted nanoscale radiation heat flux between two parallel silicon plates [3].
The difficulty in controlling a heating source can be overcome by using a heated cantilever mounted on an atomic force microscope. Heated cantilevers have been fabricated by Professor W.P. King and his group at Georgia Tech. Cantilever tips with radius of curvature as small as 20 nm can be fabricated and the tip temperature can be raised up to 1000 K. The heated tip provides a highly local heating source, whose temperature can be controlled. Moreover, the use of AFM enables the manipulation of the gap between the tip and the substrate to within a few nanometers. Accurate measurements of the nanoscale thermal radiation from a heated AFM cantilever require a sensor that can measure the temperature rise with nanometer spatial resolution. This requirement will be satisfied with a nanofabricated thermocouple, in which the wire widths are less than 100 nm. We have designed on-chip calibration structures to determine the effective Seebeck coefficient of the nanoscale temperature sensor. The heat transfer between the cantilever tip and the thermocouple as well as that between the thermocouple and the substrate will be modeled when the AFM is operated in a constant distance mode as well as in a tapping mode. The comparison of the measurement with the simulation will determine the radiation heat flux between the heated cantilever tip and the substrate surface with different materials. The success of this research will clarify the radiation energy transport mechanism at a distance below 10 nm and at above room temperature. The knowledge achieved from this study will allow the development of nanoscale devices, materials, and processes that critically depend upon near-field radiative interactions.
This work has been supported by the National Science Foundation and is collaborated with Professor W.P. King and his group.
Selected Publications
[1] Zhang, Z.M., and Park. K., 2004, “On the Group Front and Group Velocity in a Dispersive Medium upon Refraction from a Nondispersive Medium,” Journal of Heat Transfer, 126, pp. 244 – 249.
[2] Park, K., Lee, B.J., Fu, C.J., and Zhang, Z.M., 2005, “Study of the Surface and Bulk Polaritons with a Negative Index Material,” Journal of the Optical Society of America B, 22, pp. 1016-1023.
[3] Fu, C.J., and Zhang, Z.M., 2005, “Nanoscale Radiation Heat Transfer for Silicon at Different Doping Levels,” submitted to International Journal of Heat and Mass Transfer.